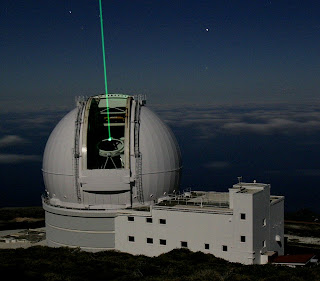
INTRODUCTION
Adaptive
optics is a new technology which is being used now a days in ground based
telescopes to remove atmospheric tremor and thus provide a clearer and brighter
view of stars seen through ground based telescopes. Without using this system,
the images obtained through telescopes on earth are seen to be blurred, which
is caused by the turbulent mixing of air at different temperatures.
Adaptive optics in effect removes this
atmospheric tremor. It brings together the latest in computers, material
science, electronic detectors, and digital control in a system that warps and
bends a mirror in a telescope to counteract, in real time the atmospheric
distortion.
The
advance promises to let ground based telescopes reach their fundamental limits
of resolution and sensitivity, out performing space based telescopes and
ushering in a new era in optical astronomy. Finally, with this technology, it
will be possible to see gas-giant type
planets in nearby solar systems in our Milky Way galaxy. Although about 100 such planets have been discovered
in recent years, all were detected through
indirect means, such as the gravitational effects on their parent stars, and
none has actually been detected directly.
ADAPTIVE OPTICS
Adaptive
optics refers to optical systems which adapt to compensate for optical effects
introduced by the medium between the object and its image. In theory a
telescope’s resolving power is directly proportional to the diameter of its
primary light gathering lens or mirror. But in practice , images from large
telescopes are blurred to a resolution no better than would be seen through a
20 cm aperture with no atmospheric blurring. At scientifically important
infrared wavelengths, atmospheric turbulence degrades resolution by at least a
factor of 10.
Space
telescopes avoid problems with the atmosphere, but they are enormously expensive
and the limit on aperture size of telescopes is quite restrictive. The Hubble
Space telescope, the world’s largest telescope in orbit , has an aperture of
only 2.4 metres, while terrestrial telescopes can have a diameter four times
that size.
In
order to avoid atmospheric aberration, one can turn to larger telescopes on the
ground, which have been equipped with ADAPTIVE OPTICS system. With this setup,
the image quality that can be recovered is close to that the telescope would
deliver if it were in space. Images obtained from the adaptive optics system on
the 6.5 m diameter telescope, called the MMT telescope illustrate the impact.
Two
images of a small region in the vicinity of the middle star in Orion’s sword- a
cluster of young stars were taken. The images show a close grouping of four
stars. In the conventional blurred image, its not possible to make out more
than two stars. With the adaptive optics on the other hand, sharpness improves
by a factor of 13, making it clear that the fainter star is ,in fact a binary –
two stars close together –and a fourth fainter member of the group appears that
was previously undetected.
BASIC PRINCIPLE
As light from a
distant star reaches the earth, it is made up of plane waves that , in the last
microseconds of their journey to the telescope, become badly distorted by
atmospheric turbulence. An adaptive optics system reflattens the wave fronts by
reflecting the light of a deformable mirror whose shape is changed in real time
to introduce an equal but opposite distortion.
The information on
how to distort the mirror comes from a wave front sensor, an instrument that
measures optical aberration imposed by the atmosphere on light from a star. A
fast computer converts the signals coming from the wave front sensor into drive
signals for the deformable mirror. The whole cycle operates at a never ending
cycle of measurement and correction, at typical speeds of 1000 updates per
second.
After the light
reflects of the deformable mirror, a beam splitter sends part of the light to a
camera that will capture the high resolution image produced by the adaptive
optics.
SEEING INFRARED
Astronomers
are mainly interested in the near and mid infrared region. A modern telescope
consists of a large concave primary mirror, designed to capture a lot of light,
and a smaller, secondary mirror that focuses the light onto a detector. In the
infrared, the hardware of adaptive optics consists of an existing telescope,
complete with its primary and secondary mirrors, and adds a separate box of
optics, including the deformable mirror, to perform the atmospheric
compensation.
This
approach has two main disadvantages. One is that each additional optical
surface beam train absorbs some of the light from the target object in the sky,
making the object appear fainter. Also it emits light by virtue of its warmth,
introducing thermal noise, further degrading the astronomers, ability to detect
faint objects.
In order to
avoid this, at the MMT, a separate secondary mirror has been built which does
double duty; it acts as a normal secondary by focusing star light onto the high
resolution imaging system, but it is also deformable, top act as the adaptive
optical wavefront corrector.
Thus
starlight coming from the telescope is already fully corrected and focuses down
to a high resolution image, with greater intensity and thermal background an
order of magnitude lower than what a telescope equipped with conventional
adaptive optics could deliver.
But
the scientific advantages of wave front correction at the telescope’s secondary
faces enormous technical challenges, the most important of them being how to
make a piece of glass whose surface could be precisely controlled and shaped to
within a few nanometers a thousand times a second.
In
order to overcome this, expertise of astronomers was called for. To make the
adaptive secondary mirror, two pieces of glass with a very low coefficient of
thermal expansion were first ground with matching spherical shapes. They were
then bonded together with a 100 micrometre thick later of pitch, a liquid that
is very viscous at room temperature.
This
arrangement holds the two pieces of glass like a single rigid body as the convex surface is ground down to
a membrane just 2 mm thick. The desired optical surface, a hyperboloid was then
polished into the membrane with the same technique used for the large primary mirrors.
To release the membrane, the whole assembly was baked to 120 degree celsius,
melting the pitch and allowing the membrane to slide off the front convex
surface of the membrane, coated with aluminium, becomes the deformable mirror.
The second
difficulty, controlling the shape of the membrane at high speed and with
extremely precision, was later solved. The problem is that the membrane is very
floppy, so that in trying to push it around to change its shape rapidly, it
rings in hundreds of resonant modes. Unchecked, these resonances would make it
possible to control the rapid changes in the shape of the mirror. but by
placing the membrane just 40 micrometre away from a second, rigid piece of
called the shape reference plate, it was discovered that the thin layer of air between them
become so viscous that all the resonances are damped out.
In
the fully assembled mirror, the membrane’s shape is controlled by 336
voice-coil actuators, like miniature loud speakers. They couple to 336
rare-earth magnets glued to the back of the membrane. The separation between
the copper coils and the magnets is 0.2 mm. A current through each coil
generates a variable magnetic field, which exerts a force on the corresponding
permanent magnet and moves the glass membrane.
Unique
to this deformable mirror are capacitive position sensors that measure the
mirror’s local position. The capacitors are chromium rings deposited on the
front surface of the reference plate around each of the 336 actuators. The
capacitance between each chromium ring and an aluminiium coating on the back of
the deformable mirror across the 40 micrometre air gap is about 65 pF. A square
wave voltage applied across the capacitors allows them to be read at 40 kHz,
giving a measure of the local position of the membrane with respect to the
rigid reference plate, accurate to 3 nm.
In
the normal orientation when installed in the telescope, the flexible membrane
is at the bottom. Above that is rigid reference plate, 50 nm thick, pierced by
336 holes through which poke the actuators. The coils of the actuators are
mounted on the ends of 10 cm long aluminium fingers that conduct heat to an
aluminium cold plate, two machined pieces glued and bolted together.
Cooling
fluid circulates through grooves milled into the lower plates. The fluid is a
mixture of distilled water and methanol. This solution won’t freeze even at
cold temperatures. Above the cold plate are three electronic units containing
168 DSPs. Each DSP is responsible for controlling two actuators, reading the
capacitive sensors and updating the drive currents in the coils to keep the
mirror in right shape. This makes it resistive to vibrations, wind buffeting
and changes in the direction of gravity. It has been shown that the MMT
adaptive optics system holds its shape to an astonishing 10nm against winds at
speeds of about 50 kmph.
Thus
with the difficulties in building an adaptive secondary mirror overcome, we
will be able to see in detail a mechanism by which stars of varying masses are
distributed through the galaxy.
Canceling
distortion in the MMT’s adaptive optics system, light from the primary mirror,
distorted by the atmosphere, reflects
from the adaptive secondary mirror that is deformed to correct for the
distortion. A beam splitter shunts some light from this mirror to a
wavefront sensor. The sensor’s output
goes to an array of digital signal processors in a control computer, which
calculates how much and where to deform the mirror to compensate for the
atmospheric distortion. The corrected light passes through a lens that focuses
it into a high-resolution image.
OTHER STARS, OTHER EARTHS
Perhaps
the most exciting scientific program to benefit from the new approach to
adaptive optics will be to look at Jupiter like planets orbiting other stars.
Roughly 100 such stars have been found out through observations of the effects
on the motion of their parent stars, but none has ever be seen by direct
imaging. It happens because they are extraordinarily faint and to compound the
problem, they are right next to something that is enormously brighter.
We
can learn about the environments in which the planets form. Measurements of the
planet’s brightness at different wavelengths will tell us about the planet’s
temperature and chemical composition and whether the system has conditions to
support life. Observations in the thermal infrared region of the spectrum will
be particularly valuable because many simple organic molecules like methane emit
strongly there. We can also exercise many of the observational techniques and
new technologies required to eventually find and study earth-like planets.
The
big challenge here is to distinguish a planet’s light from that of its parent star. In the visible range, where
planets shine by reflecting starlight, contrast ratios between a planet and its
star can be extremely large.
Younger giant planets, less than a billion
years old or so, still retain much of the heat created by their evolution out
of the primeval matter from which their solar systems were formed, and radiate
strongly in the thermal infrared. But most planetary systems, like our own are
much older. They will have cooled and will no longer glow in the thermal
infrared as they once did.
A
further complication occurs when trying to capture an image of the stellar
system at the telescope. Regions of the image close to the star, where its
planets may be found, are swamped by a halo of starlight scattered by earth’s
atmosphere. The halo adds photon noise orders of magnitude greater than the
tiny
planetary signal. To hope of finding
the planet, we must rely on adaptive optics to suppress the halo as much as
possible. The very low thermal background radiation coming from the MMT
adaptive optics system provides a crucial advantage by reducing the photon
noise against which the planet must be seen.
The
stellar halo can also be suppressed still further through destructive
interference, using a technique called nulling interferometry. In this
procedure, the two images from two telescopes are overlapped exactly and in
such a way that at the location of the star, the crests in the light waves from
one telescope fall on the troughs of the waves from the other, canceling each
other out. Thus a dark image is formed where, before, the bright stellar image
was found.
The
principle of conservation of energy requires that the starlight not be
destroyed, and indeed it appears at a second output of the nulling
interferometer. It is removed, though, in the crucial region closest to the
star we would expect to look for planets. Planetary images will therefore be
seen with greatly improved contrast.
FUTURE ENHANCEMENTS
As
we continue to develop this program, further improvements in our
instrumentation will allow us to see fainter objects. The next major step will
be the completion of the Large Binocular telescope, combining two 8.4 m primary
mirrors on a single mount , each equipped with its own secondary mirror. The
corrected light from the two halves of the telescope will then be brought
together in the centre in a new nulling interferometer which is being built.
Predictions
of the instrument’s sensitivity show that we can expect direct detection of
several planets already known to exist like Ursae Majoris, and v Andromedae. Many others are likely to be
discovered for the first time because of the instrument’s ability to explore a
much greater region of space around each star than is possible with today’s
indirect detection methods.
ADVANTAGES
1. Science does not so far have any
means of avoiding atmospheric turbulence. So it is the only method used to study
faint distant objects.
2. It greatly improves resolution of
images obtained from ground based telescopes.
3. Telescopes fitted with adaptive
optics provide an image even better than that provided by space telescopes.
4. The aperture size of ground
based telescopes can be about four times that of space telescopes.
Conclusion
There
are many substantial technological challenges in AO. Among them are the
development of fast, very low-noise detectors in order to be able to correct
with fainter reference stars; high-power reliable & easy to operate sodium
lasers; very fast processors exceeding 109 to 1010 operations per second;
deformable mirrors with bandwidths of several kilohertz and with thousands of
actuators, and large secondary adaptive mirrors. The latter are especially
interesting at thermal wavelengths, where any additional mirror raises the
already huge thermal background seen by the instruments. Many recent
astronomical discoveries can be directly attributed to new optical observation
capabilities. With the new generation of Very Large Telescopes entering into
operation, the role of AO systems is extremely important.
No comments:
Post a Comment
leave your opinion